6.1 Synaptic Transmission in a Simple Reflex Circuit
One of the simplest behaviors mediated by the central nervous system is knee-jerk or stretch reflex. In response to a neurologist's hammer to the patella tendon, there is a reflex extension of the leg. Figure 6.1 illustrates the neurocircuitry that controls that reflex response. The stretch to the patella tendon stretches the extensor muscle. More specifically, it stretches a group of specific receptors known as muscle spindle receptors or simply stretch receptors.
Figure 6.1 |
The sensory neurons also make synaptic connections with another type of neuron in the spinal cord called an interneuron. Interneurons are so named because they are interposed between one type of neuron and another. The particular interneuron shown is an inhibitory interneuron. As a result of its activation through the process of synaptic transmission, action potentials are elicited in the interneuron. An action potential in the inhibitory neuron leads to the release of a chemical transmitter substance that inhibits the flexor motor neuron, thereby preventing an improper movement from occurring. This particular reflex is known as the monosynaptic stretch reflex because this reflex is mediated by a single excitatory synaptic relay in the central nervous system.
Synaptic Potentials
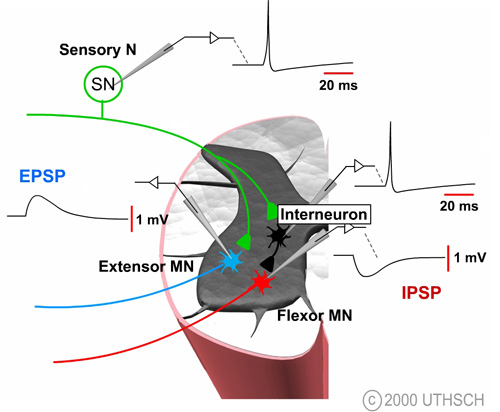
Figure 6.2
The figure at right illustrates how it is possible to experimentally examine some of the components of synaptic transmission in the reflex pathway that mediates the stretch reflex. Normally, the sensory neuron is activated by a stretch to the stretch receptor, but this process can be bypassed by injecting a depolarizing current into the sensory neuron. That stimulus initiates an action potential in the sensory neuron which leads to a change in the potential of the motor neuron. This potential is known as an excitatory postsynaptic potential (EPSP); excitatory because it tends to depolarize the cell, thereby tending to increase the probability of firing an action potential in the motor neuron and postsynaptic because it is a potential recorded on the postsynaptic side of the synapse.
The ionic mechanisms for the EPSP in the spinal motor neuron are essentially identical to the ionic mechanisms for the EPSP at the neuromuscular junction. Specifically, the transmitter substance diffuses across the synaptic cleft and binds to specific ionotropic receptors on the postsynaptic membrane, leading to a simultaneous increase in the sodium and potassium permeability (See Figure 4.10). The mechanisms for release are also identical to those at the neuromuscular junction. An action potential in the presynaptic terminal leads to the opening of voltage dependent Ca2+ channels, and the Ca2+ influx causes transmitter substance to be released.
6.3 Differences between the EPSP at the Skeletal Neuromuscular Junction and EPSPs in the CNS
There are two fundamental differences between the process of synaptic transmission at the sensorimotor synapse in the spinal cord and the process of synaptic transmission at the neuromuscular junction. First, transmitter substance released by the sensory neuron is not ACh but rather the amino acid glutamate. Indeed, there are many different transmitters in the central nervous system - up to 50 or more and the list grows every year. Fortunately, these 50 or more transmitter substances can be conveniently grouped into four basic categories: acetylcholine, monoamines, peptides, and the amino acids. Second, in contrast to the 50-mV amplitude of the synaptic potential at the neuromuscular junction, the amplitude of the synaptic potential in a spinal motor neuron, as a result of an action potential in a 1A afferent fiber, is only about 1 mV.
6.4 Temporal and Spatial Summation
If the amplitude of the postsynaptic potential is only 1 mV, how can an action potential in the motor neuron be triggered and the reflex function? Note that a 1-mV EPSP is unlikely to be sufficient to drive the membrane potential of the motor neuron to threshold to fire a spike. If there is no spike, there will be no contraction of the muscle. The answer is that the stretch of the muscle fires multiple action potentials in many different stretch receptors. In fact, the greater the stretch, the greater is the probability of activating more stretch receptors. This process is referred to as recruitment. Therefore, multiple 1A afferents will converge onto the spinal motor neuron and participate in its activation. This is not the whole answer, however. Recall that the greater the intensity of the stimulus, the greater is the number of action potentials elicited in a sensory receptor. The greater the stretch, the greater the number of action potentials elicited in a single sensory neuron and the greater number of EPSPs produced in the motor neuron from that train of action potentials in the sensory cell. The processes by which the multiple EPSPs from presynaptic neurons summate over space and time are called temporal and spatial summation.
Figure 6.3 |
Temporal summation. A single action potential in sensory neuron 1 produces a 1-mV EPSP in the motor neuron. Now consider the consequences of firing two action potentials in quick succession (See figure above). Two EPPs are elicited, the second of which summates on the falling edge of the first. As a result of two action potentials, a summated potential about 2 mV in amplitude occurs. If there were three presynaptic action potentials, and they occurred rapidly enough, the total potential would be about 3 mV, and so forth. Temporal summation is strictly a passive property of nerve cells. Special ionic conductive mechanisms are not needed to explain it. The potentials summate because of the passive properties of the nerve cell membrane, specifically the ability of membranes to store charge. The membrane temporarily stores the charge of the first PSP and then the charge from the second PSP is added to it to produce a potential twice as large at first. This process of temporal summation is very much dependent upon the duration of the synaptic potential. The temporal summation occurs when the presynaptic action potentials occur in quick succession. The time frame is dependent upon the passive properties of the membrane, specifically the time constant.
Spatial summation. Now consider a motor neuron that receives two inputs. An action potential produced in sensory neuron 1 produces a 1-mV EPSP and a single action potential in sensory neuron 2 also produces a 1-mV EPSP. If action potentials are produced simultaneously in sensory neuron 1 and in sensory neuron 2, the EPSPs summate to produce a summated EPSP which is twice that of the individual EPSPs. Spatial summation in nerve cells occurs because of the space constant, the ability of a charge produced in one region of the cell to spread to other regions of the cell.
6.5 IPSPs
Whether a neuron fires in response to a synaptic input is dependent upon how many action potentials are being fired in any one afferent input, as well as how many individual afferent pathways are activated.
The decision to fire also depends on the presence of inhibitory synaptic inputs. Artificially depolarizing the interneuron to initiate an action potential produces a transient hyperpolarization of the membrane potential of the motor neuron (See Figure 6.2). The time course of this hyperpolarization looks very similar to that of an EPSP, but it is reversed in sign. The synaptic potential in the motor neuron is called an inhibitory postsynaptic potential (IPSP) because it tends to move the membrane potential away from the threshold, thereby decreasing the probability of this neuron initiating an action potential.
6.6 Ionic Mechanisms for IPSPs
The membrane potential of the flexor motor neuron is about -65 mV, so one might predict that the IPSP would be due to an increase in the permeability or the conductance of an ion whose equilibrium potential is more negative than -65 mV. One possibility is potassium. Potassium does mediate some inhibitory synaptic potentials in the central nervous system, but not at the particular synapse between a spinal interneuron and spinal motor neuron. At this particular synapse, the IPSP is due to a selective increase in chloride permeability. Note that the equilibrium potential for chloride is about -70 mV. The transmitter released by the spinal interneuron binds to a special class of ionotropic receptors which are normally closed, but open and become selectively permeable to chloride ions as a result of the binding of the transmitter. As a result of the increase in Cl- permeability, the membrane potential moves from its resting value of -65 mV towards the Cl- equilibrium potential. (Note that in principle, decreasing the resting conductance of Na+ could also produce an IPSP.)
6.7 Transmitter Substance of the Spinal Inhibitory Neuron
What about the transmitter substance that is released by the inhibitory interneuron in the spinal cord? The transmitter substance is glycine, an amino acid which is used frequently in the central nervous system as a transmitter that produces inhibitory actions. It is not the most common, however. The most common transmitter with inhibitory actions is gamma amino butyric acid (GABA).
6.8 Metabotropic Synaptic Responses
In addition to the responses mediated by ionotropic receptors, there is an entirely separate class of synaptic potentials that have durations with orders of magnitude greater than the durations of the classical EPSPs. These are so-called slow synaptic potentials and they are mediated by metabotropic receptors. Slow synaptic potentials are not observed at every postsynaptic neuron but they are certainly observed at many. The figure below illustrates a postsynaptic neuron which receives two inputs. An action potential in neuron 1 produces an excitatory postsynaptic potential or EPSP in the postsynaptic cell whose duration is about 20 msec. Neuron 2 can also produce a postsynaptic potential but its duration is more than three orders of magnitude longer than that of the conventional type of synaptic potential. The mechanism of these slow synaptic responses involves changes in metabolism of the cell.
Figure 6.4 |
Figure 6.5 |
One mechanism for a slow synaptic potential is shown in the illustration at left (Figure 6.5) and in Figure 11.11. In contrast to the ionotropic receptor for which the receptors are actually part of the channel complex, the channels that produce the slow synaptic potentials are not directly coupled to the transmitter receptors. Rather, the receptors are separate from the channel. These receptors are known as metabotropic because they involve changes in the metabolism of the cell and, in general, changes in activation of specific second messenger systems. The figure at left illustrates an example of one type of response that involves the cyclic AMP cascade. Slow PSPs are in some cases mediated by cyclic AMP but they are also mediated by other protein kinases. For the response in Figure 6.5, the transmitter activates G proteins that lead to the increased synthesis of cyclic AMP. Cyclic AMP then leads to the activation of cyclic AMP-dependent kinase (PKA), which phosphorylates a channel protein or a component of the channel and then produces a conformational change in the channel and a change in its ionic permeability. In contrast to a direct conformational change produced by the binding of a transmitter to the receptor channel complex (seen in responses mediated by ionotropic receptors), the conformational change is produced by phosphorylation. The particular channel is one that is selectively permeable to K+ and is normally open. As a result of the channel phosphorylation by PKA, the channel closes and becomes less permeable to K+. Since the normal resting potential is due to a balance of Na+ and K+, decreasing the K+ conductance favors the effects of the Na+ conductance and a depolarization is produced.
It is interesting to point out that the activation of metabotropic receptors can produce effects which are much longer than several hundred seconds. For example, protein kinase A can diffuse in the nucleus where it can phosphorylate proteins (i.e., transcription factors) that regulate gene expression.
6.9 Types of Synaptic Transmission
This chapter and the two previous ones have focused on chemical synaptic transmission. As you have seen for chemical synapses, there is a distinct cytoplasmic discontinuity that separates the presynaptic and postsynaptic membranes (Fig. 6.6A).
Figure 6.6A |
Figure 6.6B |
This discontinuity is known as the synaptic cleft. The presynaptic terminal of chemical synapses contains a high concentration of mitochondria and synaptic vesicles, and there is a characteristic thickening of the postsynaptic membrane. As a result of a depolarization or an action potential in the presynaptic terminal, chemical transmitters are released from the presynaptic terminal, which diffuse across the synaptic cleft and bind to receptor sites on the postsynaptic membrane. This leads to a permeability change that produces the postsynaptic potential. For chemical synapses, there is a delay (usually, approximately 0.5-1 ms in duration) between the initiation of an action potential in the presynaptic terminal and a potential change in the postsynaptic cell. The synaptic delay is due to the time necessary for transmitter to be released, diffuse across the cleft, and bind with receptors on the postsynaptic membrane. Chemical synaptic transmission is generally unidirectional. A potential change in the presynaptic cell releases transmitter that produces a postsynaptic potential, but a depolarization in the postsynaptic cell does not produce any effects in the presynaptic cell because no transmitter is released from the postsynaptic cell at the synaptic region. The most predominant type of synapse is the chemical synapse, and for that reason they have been the focus of this and the previous chapters.
However, another category of synapses are those associated with electrical synaptic transmission. Electrical synaptic transmission is mediated by specialized structures known as gap junctions (Fig. 6.6B), which provide a pathway for cytoplasmic continuity between the presynaptic and the postsynaptic cells. Consequently, a depolarization (or a hyperpolarization) produced in the presynaptic terminal produces a change in potential of the postsynaptic terminal, which is due to the direct ionic pathway between the cells. For electrical synapses, a minimal synaptic delay is present; as soon as a potential change is produced in the presynaptic terminal, a reflection of that potential change is produced in the postsynaptic cell. Electrical junctions are found in both the nervous system and between other excitable membranes, such as smooth muscle and cardiac muscle cells. In these muscle cells, they provide an important pathway for the propagation of action potentials from one muscle cell to another.
The discovery of certain toxins has greatly facilitated the analysis of voltage and chemically gated channels as well as the process of synaptic transmission. The following table illustrates some that have been particularly useful.
Some Important Neurotoxins | |
tetrodotoxin (TTX) | Fish toxin that blocks the pore of voltage-dependent Na+ channels. |
μ-conotoxin (μ-CTX) |
Fish-hunting cone snail toxin with properties similar to TTX. |
saxitoxin (STX) | Toxin from marine dinoflagellates with properties similar to TTX. STX is also known as paralytic shellfish poison. |
ω-conotoxin (ω-CTX) |
Fish-hunting cone snail toxin that blocks certain types of voltage-dependent Ca2+ channels. |
funnel web spider toxin (ω-Aga) | Toxin from funnel web spider which blocks certain types of voltage-dependent Ca2+ channels. |
apamin |
Bee venom toxin that blocks certain types of Ca2+-activated K+ channels. |
charybdotoxin (ChTX) | Scorpion venom toxin that blocks pore of some Ca2+-activated K+ channels and voltage-dependent K+ channels. |
curare (d-tubocurarine) |
Plant toxin that is a competitive inhibitor of nicotinic ACh receptors. |
α-bungarotoxin | Snake toxin that is competitive and highly irreversible inhibitor of nicotinic ACh receptors. |
picrotoxin | GABAA receptor blocker isolated from the seed of Anamirta cocculus. |
strychnine | Glycine receptor blocker isolated from the seed of the East Indian tree Strychnos nux-vomica. |
tetanus toxin |
Clostridial neurotoxin with zinc-dependent protease activity; Cleaves synaptic vesicle proteins in the CNS and thereby blocks release of neurotransmitters. |
botulinum toxin | Clostridial neurotoxin with zinc-dependent protease activity; Cleaves synaptic vesicle proteins at the neuromuscular junction and thereby blocks release of ACh. |